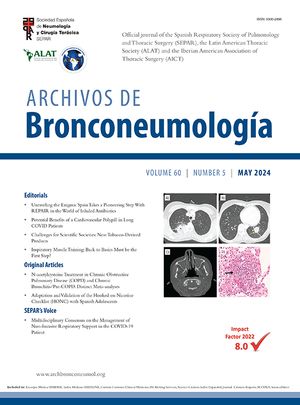
With the current COVID-19 pandemic, concerns have raised regarding the risk for NIV to promote airborne transmission. In case of hospital admission, continuation of therapy in patients undergoing chronic NIV is necessary and several protective circuit configurations have been recommended to reduce the risk of aerosol dissemination. However, all these configurations increase instrumental dead space. We therefore designed this study to evaluate their effects on the tidal volume (VTE) required to preserve stable end-tidal CO2 partial pressure (PETCO2) with constant respiratory rate.
MethodsA bench consisting of a test lung connected to an adult-sized mannequin head was set up. The model was ventilated through usual domiciliary configuration (single limb circuit with facial vented mask) which was used as reference. Then, five different circuit configurations including non-vented facial mask with viral/bacterial filter, modification of leak position, and change from single to double-limb circuit were evaluated. For each configuration, pressure support (PS) was gradually increased to reach reference PETCO2. Resulting VTE was recorded as primary outcome.
ResultsReference PETCO2 was 38(0)mmHg, with a PS set at 10cmH2O, resulting in a VTE of 432(2)mL. Compared to reference, all the configurations evaluated required substantial increase in VTE to preserve alveolar ventilation, ranging from +79(2) to +216(1)mL.
ConclusionsModifications of NIV configurations in the context of COVID-19 pandemic result in substantial increase of instrumental dead space. Re-evaluation of treatment efficiency and settings is crucial whenever protective measures influencing NIV equipment are considered.
Durante la actual pandemia de COVID-19 ha surgido la preocupación sobre el posible riesgo de que la ventilación no invasiva (VNI) promueva la transmisión aérea. En el caso de ingreso hospitalario, es necesario continuar con el tratamiento de aquellos pacientes tratados con VNI crónica y se han recomendado varias configuraciones protectoras de los circuitos para reducir el riesgo de diseminación por aerosoles. Sin embargo, todas estas configuraciones aumentan el espacio muerto instrumental. Así, diseñamos este estudio para evaluar los efectos de estas configuraciones sobre el volumen corriente (VCE) necesario para mantener estable la presión parcial de CO2 al final del volumen corriente espirado (PETCO2) con una frecuencia respiratoria constante.
MétodosSe construyó un modelo experimental que constaba de un pulmón de prueba conectado a una cabeza de maniquí de tamaño adulto. El modelo recibió ventilación utilizando la configuración domiciliaria habitual (circuito de rama única con máscara facial ventilada), lo que se utilizó como referencia. Después se evaluaron cinco configuraciones diferentes del circuito, incluidas la máscara facial sin ventilación con filtro antiviral/antibacteriano, la modificación de la posición de la fuga y el cambio de circuito de rama única a doble rama. Para cada configuración, la presión de soporte (PS) se incrementó gradualmente hasta alcanzar la PETCO2 de referencia. El VCE resultante se registró como resultado primario.
ResultadosLa PETCO2 de referencia fue de 38(0)mmHg, con una PS fijada en 10cmH2O, lo que resultó en un VCE de 432(2)mL. En comparación con la referencia, todas las configuraciones evaluadas requirieron un aumento sustancial del VCE para preservar la ventilación alveolar, en un rango entre +79(2)mL y +216(1)mL.
ConclusionesLas modificaciones de las configuraciones de VNI en el contexto de la pandemia de COVID-19 resultan en un aumento sustancial del espacio muerto instrumental. Reevaluar la eficacia y los ajustes del tratamiento es fundamental cuando se ponen en consideración unas medidas de protección que influyen en el equipo de VNI.
Non-invasive ventilation (NIV) is the standard of care for the treatment of chronic hypercapnic respiratory failure.1 It can be conducted through different configurations, single-limb circuits with facial masks being the most frequently met in the home setting.1 This implies the presence of an intentional leak either at the mask or between the mask and circuit, preventing exhaled air to be rebreathed. However, substantial dissemination of exhaled particles into patients’ environment has been documented.2
With the current COVID-19 pandemic, growing interest regarding the risk for NIV to promote airborne transmission has emerged.3 This concern has led by itself to discuss the indication of this therapy in patients requiring hospital admission for de novo SARS-CoV-2 infection or in situations of acute-on-chronic respiratory failure.4–6 In the latter situation, patients treated with chronic NIV may worsen with ventilation cessation and protective measures allowing for pursuing therapy in and out of the hospital setting are necessary.
Thus, several adaptations of circuit configuration in order to reduce the risk of NIV-related viral contaminations have been proposed worldwide.7–11 Most of these recommendations share the common aspect that they increase instrumental dead space (VD) and airway resistance compared to “usual” configuration. As a consequence, any modification of NIV circuit configuration when applying these protective recommendations may require NIV settings adjustments to preserve ventilation efficiency.
We therefore designed this bench study in order to evaluate the effects of circuit configurations recommended for NIV during COVID-19 pandemic on the pressure support (PS) and expired tidal volume (VTE) modifications required to maintain treatment efficiency with respect to end-tidal CO2 partial pressure (PETCO2).
MethodsRespiratory System ModelA test lung was used to simulate the patient's respiratory mechanics (compliance: 60mL/cmH2O, resistance: 5cmH2O/L/s). These parameters were chosen to be central enough to be consistent both with respiratory mechanics of patients undergoing chronic NIV and patients eligible for NIV at hospital admission in the context of suspected SARS-CoV-2 infection.12 In order to simulate CO2 production, a constant flow of 100% CO2 (V′CO2) was provided into the test lung (180±5mL/min).13
Ventilation of the test lung was performed with the Astral 150 (SR6; ResMed Ltd, Bella Vista, NSW, Australia) set with PS 10cmH2O and expiratory positive airway pressure (EPAP) 6cmH2O. Rise time was set to 150ms, trigger medium, cycling 50% of peak inspiratory flow, minimum and maximum inspiratory times (TiMin and TiMax) set to offer a window from 1/4 to 1/2 of total breath cycle time, i.e. 0.6 and 1.2s, respectively. The simulated patient was passively ventilated and respiratory rate was driven by the backup rate, set at 25bpm.
Data AcquisitionRespiratory flow (V′aw), airway pressure (Paw) and PCO2 were continuously monitored between the mannequin head and the test lung (Supplementary Fig. 1). Partial pressure of end-tidal CO2 (PETCO2) was defined as the maximum PCO2 value reached at the end of expiration. Steady state was determined as a period of at least 5min of stable ventilation assessed by visual inspection of respiratory flow and pressure curves, and stable PETCO2 (i.e. variations<0.5mmHg from breath to breath).
Data were analysed with a dedicated software. Inspiratory positive airway pressure measured between the mannequin and the test lung (IPAPaw) was determined as the maximum Paw reached during inspiratory time; positive end-expiratory pressure (PEEPaw) was determined as mean Paw during the last 200ms of expiratory time. Inspiratory time (Ti) was defined as the duration in seconds from the onset of positive V′aw to the onset of negative V′aw. Peak inspiratory flow (PIF) was determined as the maximal V′aw value reached during inspiratory time. Expired tidal volume (VTE) was calculated as the integral of V′aw over expiratory time. Additional measurements were performed to determine circuit compliance and resistances according to the different configurations evaluated, as detailed in the supplementary material.
ProtocolConfiguration A consisted in a single-limb circuit (Standard air tubing, ResMed Ltd, Bella Vista, NSW, Australia) and a facial mask with built-in intentional leak (Quattro Air – Medium size, ResMed Ltd, Bella Vista, NSW, Australia).1 The mask was carefully fitted on the mannequin head to avoid non-intentional leaks. Calibration of the device and circuit up to the end of the tubing was performed prior to data acquisition and remained unmodified throughout the protocol except for configurations E and F (double-limb circuit). Configuration B consisted in a serial connection of circuit (Standard air tubing, ResMed Ltd, Bella Vista, NSW, Australia), calibrated intentional leak (ResMed leak valve, ResMed Ltd, Bella Vista, NSW, Australia), viral/bacterial filter (Air-Guard™ Clear, Intersurgical Ltd, UK, internal volume: 120mL), and non-vented facial mask (Quattro Air NV – Medium size, ResMed Ltd, Bella Vista, NSW, Australia).7 In configuration C the circuit (Standard air tubing, ResMed Ltd, Bella Vista, NSW, Australia) was connected to the mask (Quattro Air NV – Medium size, ResMed Ltd, Bella Vista, NSW, Australia) via a T-connector, to the remaining port of which was connected the filter (Air-Guard™ Clear, Intersurgical Ltd, UK), followed by the leak (ResMed leak valve, ResMed Ltd, Bella Vista, NSW, Australia) and an occlusion cap (Intersurgical Ltd, UK).8 Configuration D consisted in configuration B with a 15cm flexible tubing with 22mm inner diameter inserted between the filter and the leak.9 Configuration E consisted in a double-limb circuit (Smoothbore breathing system, Intersurgical Ltd, UK), connected to a non-vented facial mask (Quattro Air NV – Medium size, ResMed Ltd, Bella Vista, NSW, Australia), with two filters (Air-Guard™ Clear, Intersurgical Ltd, UK) placed both at the inspiratory and expiratory port of the ventilator.10 Configuration F consisted in configuration E with a filter placed between the Y-piece and the mask.11
First, a baseline assessment was performed with the usual domiciliary configuration (Fig. 1, upper panel; configuration A). After steady state was reached, PETCO2 was recorded and used as reference for the following experiments. Then, five additional configurations recommended for NIV during COVID-19 pandemic were successively evaluated (Fig. 1, upper panel; configurations B–F). With each of these configurations, resulting PETCO2 and VTE with baseline pressure support (10cmH2O) were recorded (iso-PS variables).
PS and VTE variations required to maintain iso-PETCO2 according to the configurations evaluated.
Upper panel: configuration A: conventional domiciliary configuration; configuration B: from Ref. 7; configuration C: from Ref. 8; configuration D: from Ref. 9; configuration E: from Ref. 10; configuration F: from Ref. 11; for configurations E and F, two additional filters were placed both at the inspiratory and expiratory port of the ventilator (not shown).
Lower panel: data are expressed as mean (SD). ΔPS (cmH2O, dashed line), difference between pressure support set for a given configuration and reference PS (configuration A; 10cmH2O). ΔVTE (mL, solid line): difference between measured VTE for a given configuration and reference VTE (configuration A; 432mL). *Clinically relevant variation compared to reference (configuration A). Note that ΔVTE represents the increase in VTE required to preserve alveolar ventilation with constant respiratory rate, i.e. the additional instrumental dead space in mL.
For each configuration, PS was manually incremented to reach reference PETCO2 (configuration A), and therefore to determine the tidal volume variations required to maintain isocapnia (i.e. stable alveolar ventilation). The additional VD with the different configurations evaluated was calculated according to Bohr's equation (see supplementary material). PETCO2 deviation from reference <1mmHg was considered clinically acceptable. Data analysis was performed with a dedicated software on a breath by breath basis over 1min of stable ventilation. Mean and standard deviation (SD) were calculated for each variable of each configuration and used for between-groups comparison.
Statistical AnalysisVTE difference (ΔVTE) between reference configuration (A) and other configurations (n) was calculated as VTE(n)−VTE(A) and absolute value expressed in mL was used for analysis. The same calculation was performed to determine pressure support and dead space differences (respectively, ΔPS; in cmH2O, and ΔVD; in mL) between reference and other configurations. Data were described as mean (SD). Given the simulation-based design of this study, standard variations for each variable was extremely small and any statistical analyses would have yielded significant results. Therefore, we arbitrarily chose a cut off set at ±10% above which variations from reference configuration's mean±SD (config. A) would be considered clinically relevant.
Additional information regarding the methods is provided in the supplementary material.
ResultsFor configuration A, reference PETCO2 at steady state was 38(0)mmHg, with a PS set at 10cmH2O resulting in a VTE of 432(2)mL. With the baseline ventilatory parameters, substantial variations in VTE were observed, associated with a clinically relevant increase in PETCO2 (Table 1; iso-PS variables). Consequently, all the configurations evaluated required higher PS to maintain stable PETCO2 (Table 1; iso-PETCO2 variables). V′CO2 remained within clinically acceptable range (180(5)mL/min) throughout the protocol. The absolute increase in PS (ΔPS, cmH2O) to maintain iso-PETCO2 for each configuration is depicted in Fig. 1, lower panel. This resulted in higher VTE for all configurations evaluated compared to reference configuration (Fig. 1, lower panel), respectively +142(7), +91(1), +216(1), +79(2) and +144(7)mL with configurations B–F. The calculation of additional VD according to Bohr's equation yielded similar results, with an increase in VD by +143(7), +91(2), +216(2), +81(3) and +148(6)mL with configurations B–F, respectively. Ventilatory parameters measured in the condition of stable alveolar ventilation (iso-PETCO2) are displayed in Table 2. The time course of Paw, V′aw and PCO2 for the reference configuration (A) and the configuration requiring the maximum PS and VTE modifications to reach reference PETCO2 (configuration D) is depicted in Fig. 2.
Iso-PS and Iso-PETCO2 Basic Monitoring According to the Different Configurations Evaluated.
Variables | Config. A | Config. B | Config. C | Config. D | Config. E | Config. F |
---|---|---|---|---|---|---|
Iso – PS | ||||||
Settings (PS/PEEP), cmH2O | 10/6 | 10/6 | 10/6 | 10/6 | 10/6 | 10/6 |
PETCO2, mmHg | 38 (0) | 93 (1)* | 64 (1)* | 99 (0)*,a | 53 (1)* | 89 (1)* |
VTE, mL | 432 (2) | 379 (1)* | 413 (2) | 383 (2)* | 449 (2) | 394 (1) |
Iso – PETCO2 | ||||||
Settings (PS/PEEP), cmH2O | 10/6 | 19/6* | 14/6* | 22/6* | 13/6* | 18/6* |
PETCO2, mmHg | 38 (0) | 38 (0) | 38 (1) | 37 (0) | 38 (0) | 39 (0) |
VTE, mL | 432 (2) | 574 (7)* | 523 (1)* | 648 (1)* | 511 (2)* | 576 (7)* |
Iso-PS variables were recorded with PS set at 10cmH2O. Iso-PETCO2 variables were recorded with PETCO2 set at 38(1)mmHg.
Results were obtained with respiratory rate set at 25breaths/min and V′CO2 set at 180(5)mL. Other ventilatory settings remained unchanged for the various configurations evaluated.
Data are presented as mean (SD).
PS, pressure support; PEEP, positive end-expiratory pressure; PETCO2, end-tidal CO2 partial pressure; VTE, expired tidal volume.
Ventilatory Parameters Measured in the Condition of Stable Alveolar Ventilation According to the Different Configurations Evaluated.
Variables | Config. A | Config. B | Config. C | Config. D | Config. E | Config. F |
---|---|---|---|---|---|---|
IPAPaw, cmH2O | 16.7 (0.0) | 22.8 (0.3)* | 19.8 (0.0)* | 26.3 (0.0)* | 19.0 (0.0)* | 22.4 (0.3)* |
PEEPaw, cmH2O | 7.4 (0.1) | 8.8 (0.0)* | 8.0 (0.0) | 9.5 (0.0)* | 8.5 (0.2)* | 9.6 (0.3)* |
PIF, L/min | 32 (1) | 38 (1)* | 36 (1) | 43 (1)* | 35 (1) | 36 (1) |
Ti, s | 1.1 (0.0) | 1.2 (0.0) | 1.2 (0.0) | 1.2 (0.0) | 1.2 (0.0) | 1.2 (0.0) |
Results were obtained with respiratory rate set at 25 breaths/min, PETCO2 set at 38(1)mmHg and V’CO2 set at 180(5)mL.
Data are presented as mean (SD).
IPAPaw, inspiratory positive airway pressure; PEEPaw, airway positive end-expiratory pressure; PIF, peak inspiratory flow; Ti, inspiratory time.
Time course of Paw, V′aw and PCO2 for configurations A and D.
Paw, airway pressure; V′aw, respiratory flow; PCO2, partial pressure of CO2. Vertical purple lines: solid line, beginning of inspiration; dashed line, end of inspiration. The figure illustrates the need to increase pressure support and thus tidal volume to maintain isocapnia (38(1)mmHg). Note that the duration of PCO2 decline during inspiration in configuration D is much longer than configuration A, suggesting higher volume of CO2 rebreathed from additional instrumental dead space.
Compared to reference (config. A), circuit compliance was increased with all the configurations evaluated (see supplementary Table 1). Mean compliance ranged from 0.4 to 2.2mL/cmH2O with configurations A–F, respectively. At 0.5L/s, the lowest (1.4cmH2O/L/s) and highest (3.3cmH2O/L/s) inspiratory resistances were encountered with configurations C and F, respectively. Configurations B, C and D resulted in substantially higher expiratory resistances than configurations A, E and F (see supplementary Table 1), with the lowest (2.2cmH2O/L/s) and highest (20.0cmH2O/L/s) values encountered with configurations E and D, respectively. Supplementary Fig. 2 displays the pressure–flow relationship for each configuration during inspiration and expiration.
DiscussionIn this study, we demonstrated that substantial adjustments in NIV settings are needed to maintain alveolar ventilation when modifications of circuit configuration are required to prevent NIV-related contaminations in the current context of COVID-19 pandemic. This is particularly relevant in patients chronically ventilated pursuing their therapy at home, or exhibiting acute-on-chronic exacerbation requiring hospital admission.7,10,14 Of note, even the change from configuration A–E which is often made when patient's condition requires switching to a life-support ventilator needed setting adjustments.
In contrast, this finding is less relevant in patients for whom NIV is initiated during an acute episode because treatment efficiency can be expected, in this context, to be carefully monitored and settings adapted whenever necessary. Moreover, in patients hospitalised for an acute lung injury related to coronavirus, the initiation of bilevel NIV is highly controversial.15–17 Most guidelines recommend NIV in patients with SARS-CoV-2 infection as a “default” option when oxygen therapy or constant positive airway pressure (CPAP) are not sufficient to improve patient's status, provided that appropriate personal protective equipment is available.10,14,18,19 Its indications in this context therefore remain restricted to situations in which access to invasive mechanical ventilation is limited, in patients not eligible to ICU admission, or in patients with underlying respiratory disease or previously undergoing long-term NIV in which NIV cessation could worsen even more their condition and for whom the results of the present study apply as well.14,20
Unexpectedly, we found discrepancies between values set on the ventilator and values measured between the mannequin head and the test lung even for baseline configuration (A) in which PEEPaw was 1.4cmH2O above EPAP setting. Of note, as most ventilators do not control the pressure directly inside the mask, any additional material increasing resistances between the point of pressure measurement and the mask may affect the delivered pressure inside the mask during NIV. Advices from ventilators’ manufacturers could be helpful in daily practice when unusual circuit configurations are considered in patients undergoing NIV.
In our experimental setup, the increased PEEPaw could at least in part be explained by the elevated RR (25bpm), which however is in line with clinical characteristics of severe patients and may promote dynamic hyperinflation (Fig. 2).21 This phenomenon was even more pronounced with other configurations (>3.5cmH2O with configurations D and F). From a theoretical point of view, increased expiratory resistances related to additional equipment (filters, tubing) might explain this observation. However, the highest PEEPaw monitored for all configurations was encountered with configuration F which actually was one of the configurations providing the lowest expiratory resistance (Supplementary Fig. 2). Conversely, configuration D yielded similar PEEPaw as configuration F, despite substantially higher expiratory resistances. It should be noted that for single with leak circuits (configurations A, B, C and D), expiratory resistances were measured with the circuit closed at ventilator's side, so that exhalation could only occur through the intentional leak. This may not accurately reflect clinical situations in which an important proportion of expired tidal volume may occur inside the circuit despite the presence of an intentional leak and contribute both to increasing CO2 rebreathing and reducing expiratory resistance as previously demonstrated.22 Either way, a simple regression analysis showed a significant linear relationship between tidal volume and PEEPaw (R2=0.76 and P=.014) whereas no relationship was detected between expiratory resistance and PEEPaw. This suggests that increased PEEPaw observed in the condition of iso-PETCO2 was most likely driven by the increase in VT (additional instrumental dead space) contributing, along with high respiratory rate, to hyperinflation of the model, rather than variations in circuit resistances.
Our study has several limitations.
First, in our experimental setup, driving pressure was generated by the device solely. Simulating patient's effort would have interestingly given the opportunity to evaluate the effects of circuit configurations with CPAP mode and to assess the variations in respiratory effort required to preserve alveolar ventilation in these different situations. Unfortunately, using one chamber of the Michigan test lung as a driving chamber to simulate patient's effort would have substantially reduced the end-expiratory lung volume of the model. With clinically relevant V′CO2, a parallel connection of the two chambers of the test lung was required to reach relevant PETCO2. Another limitation to the evaluation of CPAP mode is that this study aimed to test all the circuits modifications proposed in the literature to prevent coronavirus contamination in subjects chronically ventilated, both with single and double limb circuits. Almost all adult patients treated with CPAP mode in the context of domiciliary usage are treated with devices with single with leak configuration only. The external validity of evaluating double limb circuit modifications (configurations E and F) in subjects undergoing long term CPAP would have been broadly questionable. Additionally, even though the use of CPAP mode has been widely described in COVID-19 patients,19,21 this ventilatory mode with constant pressure would not have allowed to determine the variations of driving pressure required to maintain isocapnia with respect to the design of our bench and would also not have reflected the population of interest of our study. The results of the present study therefore do not apply to this mode. Finally, we therefore decided to use a controlled ventilation condition without patient effort in order to simplify the model, considering that modifications observed in this context were only attributable to the modifications of instrumental dead space and resistances that we wanted to evaluate. Consequently, we adjusted PS according to PETCO2. In clinical practice it can be expected that patients, as long as their muscle capacities allows to do so, would increase their work of breathing – and obviously V′CO2 – in order to regulate PCO2, as previously demonstrated with heat and moisture exchangers.23 Instead of continuous monitoring of PETCO2, adjusting PS according both to clinical signs of respiratory effort and to tidal volume would be less cumbersome and more appropriate in clinical routine.24 Nevertheless, the aim of our bench study was limited to a simple comparison in tidal volumes keeping PETCO2, respiratory rate and V′CO2 constant in order to consider that, in these conditions, the tidal volume differences would therefore represent the instrumental dead space differences according to Bohr's equation.25
Second, we used a viral/bacterial filter with a large internal volume (120mL). Even though this reference was found to be used by several other teams, such large internal volume deserves discussion.8,26 Along with the use of a non-vented mask, this resulted in an increase in VTE required to reach isocapnia, i.e. in an increase of instrumental dead space, of 142(7) and 144(7)mL with configurations B and F, respectively, and in a PS (and in our situation driving pressure) nearly twice as big as with configuration A. Of note, because of fluid dynamics variations depending on circuit configuration (and particularly on the position of intentional leak), the internal volume of instrumental dead space in mL may not accurately reflect the absolute value of additional dynamic dead space as previously demonstrated by Saatci et al.27 Nevertheless, as far as equipment is available, any reduction in instrumental dead space should be encouraged in order to reduce respiratory effort and prevent patient self-inflicted lung injury (P-SILI), especially in the context of acute lung injury related to COVID-19.28
ConclusionLarge differences in additional VD exist between the recommended configurations. It is noteworthy that the configuration which induces the lowest increase of VD with single-limb circuit is configuration C, which proposes to laterally position the leak and filter with a T-piece placed immediately after the mask.8 This allows a bypass where the expiratory gas is blown in this lateral space, preventing from its reinhalation during the next insufflation. The need to re-evaluate treatment efficiency and settings is crucial whenever protective measures influencing NIV equipment are considered.
Authors’ ContributionsAll authors contributed substantially to study conception and design; MD, KL, BL and FL contributed to data acquisition; MD, GB, BL and FL contributed to data analysis and interpretation; MD, GB, ML, BL and FL drafted the manuscript. All authors revised the manuscript for important intellectual content, approved the final version, and agree to be accountable for all aspects of the work in ensuring that questions related to the accuracy or integrity of any part of the work are appropriately investigated and resolved.
FundingNone declared.
Conflict of InterestsMD is a former employee of ResMed SAS, which had no involvement in the current study. Other authors have no conflicts of interest to disclose.
The authors would like to thank Fatima Lebret and Adrian Morales Robles for their helpful contribution to Spanish translation.